2023
In 2023, our research group has made further substantial steps towards developing elementary hardware elements for quantum information processing. These elements are solid-state quantum bits, in most cases point defects which show magneto-optical behavior which can be harnessed to store and process quantum information. A proper investigation of such systems include their precise physical description, as for both their actual molecular structure and electronic system properties, as well as proposing a set of so-called quantum protocols which make them really usable as quantum bits, and finally validating their fundamental chemical and engineering properties for the usage in real quantum information processing environments. In almost every case, these systems are also promising candidates for nanometrology (nanosensing, biosensing) applications, as the physics of these two fields are closely related. We executed ab initio calculations on modeling the qubits by KIFÜ high-performance computing units as well as our local high performance computation cluster.
Formation of G-center in silicon. Thermal equilibrium is reached when the system assumes its lowest energy. This can be hindered by kinetic reasons; however, it is a general assumption that the ground state can be eventually reached. Here, we show that this is not always necessarily the case. Carbon pairs in silicon have at least three different configurations, one of them (B-configuration) is the G photoluminescence center. Experiments revealed a bistable nature with the A-configuration (Fig. A). Electronic structure calculations predicted that the C-configuration is the real ground state; however, no experimental evidence was found for its existence. Our calculations show that the formation of the A- and B-configurations is strongly favored over the most stable C-configuration which cannot be realized in a detectable amount before the pair dissociates. Our results demonstrate that automatized search for complex defects consisting of only the thermodynamically most stable configurations may overlook key candidates for quantum technology applications.
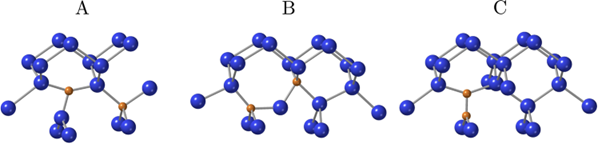
Fig. A. A, B, and C label the three configurations identified in prior studies. Brown and blues spheres are the carbon and silicon atoms, respectively. The figures are made by Crystal MakerTM.
Diamond NV center spin-phonon interactions. Spin-lattice relaxation within the diamond nitrogen-vacancy (NV) center’s electronic ground-state spin triplet limits its coherence times, and thereby impacts its performance in quantum applications. Our collaborators observed the relaxation rates on the NV center’s |ms=0⟩↔|ms=±1⟩ and |ms=−1⟩↔|ms=+1⟩ transitions as a function of temperature from 9 to 474 K in high-purity samples. We showed that the temperature dependencies of the rates are reproduced by our ab initio theory of Raman scattering due to second-order spin-phonon interactions which is generally applicable to other defect spin systems [B]. We developed a theory about the nuclear spin relaxation under resonant excitation to polarize the 14N host, which our collaborator then prove beneficial for spin magnetometry in experiments [C]. The nuclear spin relaxation is mediated by the electron-phonon coupling in the electronic excited state according to our theory.
Diamond NV centers close to the surface.
Near-surface negatively charged nitrogen vacancy (NV) centers hold excellent promise for nanoscale magnetic imaging and quantum sensing. However, they often experience charge-state instabilities, leading to strongly reduced fluorescence and NV coherence time, which negatively impact magnetic imaging sensitivity. This occurs even more severely at 4 K and ultrahigh vacuum (UHV, p = 2 × 10–10 mbar). Our collaborators demonstrate that in situ adsorption of H2O on the diamond surface allows the partial recovery of the shallow NV sensors. Our band-bending calculations showed that controlled surface treatments are essential for implementing NV-based quantum sensing protocols under cryogenic UHV conditions [D]. Molecules or ions with unpaired electronic spins are typically probed by their influence on the NV center’s spin relaxation. Whereas it is well-known that paramagnetic ions reduce the NV center’s relaxation time (T1), our collaborators reported on the opposite effect for diamagnetic ions. To elucidate the underlying mechanism of this surprising effect, single and double quantum NV experiments are performed, which indicate a reduction of magnetic and electric noise in the presence of diamagnetic electrolytes. Our ab initio simulations shows that a change in the interfacial band bending due to the formation of an electric double layer leads to a stabilization of fluctuating charges at the interface of an oxidized diamond [E].
Hexagonal boron nitride boron-vacancy spin’s coupling to external fields. Hexagonal boron nitride (hBN) has recently been demonstrated to contain optically polarized and detected electron spins that can be utilized for implementing qubits and quantum sensors in nanolayered-devices. The boron-vacancy spin defect in hexagonal boron nitride (hBN) has a great potential as a quantum sensor in a two-dimensional material that can directly probe various external perturbations in atomic-scale proximity to the quantum sensing layer. Here, we apply first-principles calculations to determine the coupling of the electronic spin to strain and electric fields. Our work unraveled the interplay between local piezoelectric and elastic effects contributing to the final response to the electric fields. The theoretical predictions are then used to analyze optically detected magnetic resonance (ODMR) spectra recorded on hBN crystals containing different densities of centers. We prove that the orthorhombic zero-field splitting parameter results from local electric fields produced by surrounding charge defects [F]. These results explain the observations of boron-vacancy spin defects in few-atomic-layers thick hexagonal boron nitride [G].
References:
[A] https://www.nature.com/articles/s41467-023-36090-2
[B] https://journals.aps.org/prl/abstract/10.1103/PhysRevLett.130.256903
[C] https://journals.aps.org/prl/abstract/10.1103/PhysRevLett.131.236901
[D] https://pubs.acs.org/doi/10.1021/acs.nanolett.2c04733
[E] https://pubs.acs.org/doi/10.1021/acsnano.3c01298
[F] https://www.nature.com/articles/s41524-023-01111-7
[G] https://journals.aps.org/prl/abstract/10.1103/PhysRevLett.131.116902